Energetically modified cement

Energetically modified cements (EMCs) are a class of cements made from pozzolans (e.g. fly ash, volcanic ash, pozzolana), silica sand, blast furnace slag, or Portland cement (or blends of these ingredients).[1] The term "energetically modified" arises by virtue of the mechanochemistry process applied to the raw material, more accurately classified as "high energy ball milling" (HEBM). At its simplest this means a milling method that invokes high kinetics by subjecting "powders to the repeated action of hitting balls" as compared to (say) the low kinetics of rotating ball mills.[2] This causes, amongst others, a thermodynamic transformation in the material to increase its chemical reactivity.[3] For EMCs, the HEBM process used is a unique form of specialised vibratory milling discovered in Sweden and applied only to cementitious materials, here called "EMC Activation".[4]
By improving the reactivity of pozzolans, their strength-development rate is increased. This allows for compliance with modern product-performance requirements ("technical standards") for concretes and mortars. In turn, this allows for the replacement of Portland cement in the concrete and mortar mixes. This has a number of benefits to their long-term qualities.[3]
Energetically modified cements have a wide range of uses. For example, EMCs have been used in concretes for large infrastructure projects in the United States, meeting U.S. concrete standards.[5]
Justification
[edit]
The term "energetically modified cement" incorporates a simple thermodynamic descriptor to refer to a class of cements produced using a specialised highly intensive milling process first discovered in 1993 at Luleå University of Technology (LTU) in Sweden.[6][7] The transformatory process is initiated entirely mechanically as opposed to heating the materials directly.[7][8][9] The mechanisms of mechanochemical transformations are often complex and different from "traditional" thermal or photochemical mechanisms.[10][11] HEBM can transform both the physical and thermodynamic properties that for example, "can lead to glass formation from elemental powder mixtures as well as by amorphization of intermetallic compound powders".[12] The effects of HEBM-transformation cause a thermodynamic change that resides ultimately in a modified Gibbs Energy.[13] The process increases the binding capacity and chemical reactivity rates of the materials transformed.[4][14]
Continuing academic work and research regarding "self-healing" properties of energetically modified cements is ongoing at LTU.[15] For example, EMCs has received awards from the Elsa ō Sven Thysells stiftelse för konstruktionsteknisk forskning (Elsa & Sven Thysell Foundation for Construction Engineering Research) of Sweden.[16] The contribution of EMCs to the domain of mechanochemistry itself has also been recognised.[17]
Etymology
[edit]The term "energetically modified cement" was first used in 1992 by Vladimir Ronin, introduced in a paper by Ronin et al. dated 1993 and presented at a formal meeting of the academic Nordic Concrete Research group.[18] The process was refined by Ronin and others, including Lennart Elfgren (now Professor Emeritus of LTU, Department of Civil, Environmental and Natural Resources Engineering).[19] In 2023, LTU awarded Elfgren the "Vice-Chancellor's Medal for Merit for outstanding and meritorious work" by virtue of his work "...for the spread of new knowledge and understanding of, in particular, the concrete construction field".[20]
At the 45th World Exhibition of Invention, Research and Innovation, held in 1996 in Brussels, Belgium, EMC Activation was awarded a gold medal with mention by EUREKA, the European inter-governmental (research and development) organisation, for "modification énergique de ciments".[21]
The term "energetically modified" has been used elsewhere—for example as recently as 2017—although such usage does not denote the method used was EMC Activation as defined here.[22]
Overview
[edit]The claims made include:[5][23][24][25][26][27]
- An EMC is a fine powder (typical of all cements) whose colour depends on the material processed.
- EMCs are produced using only a "fraction" of the energy used in Portland cement production (claimed ~100 KWh/tonne, <8% of Portland cement).
- No CO2 is released by the process. It is "zero emissions".
- The purpose of an EMC is to replace the Portland cement requirement in the mortar or concrete being used. More than 70% replacement is claimed.
- EMC Activation is a dry process.
- No noxious fumes are released.
- EMC Activation is a low temperature process, even though temperatures can be "momentarily extreme" at "sub-micron" scales.
- EMCs require no chemicals for their thermodynamic transformation.
- There are several types of EMCs, depending on the raw materials transformed.
- Depending on user-requirements, delivered dry products may comprise also a minority proportion of "high clinker" Portland cement.
- Each type of EMC has its own performance characteristics, including mechanical load and strength development. Concretes cast from EMCs may yield significant "self-healing" capabilities.
- The most frequently used EMCs are made from fly ash and natural pozzolans. These are relatively abundant materials, and the performance characteristics can exceed those of Portland cement.
- In 2009, fly ash EMCs were demonstrated to exceed the 'Grade 120 Slag' benchmark per ASTM C989 — the most reactive form of cementitious blast furnace slag.
- Silica sand and granite can also be treated by the process to replace Portland cement.
- EMC products have been extensively tested by independent labs and certified for use by several US DOTs including in Federal Highway Administration projects.
- EMCs comply with respective technical standards, such as ASTM C618-19 (U.S.); EN-197, EN-206 and EN 450-1:2012 (CEN territories, including EEA); BS 8615‑1:2019 (U.K.).
- Compared to using Portland cement, the resulting concrete-mix using EMC does not require a higher "total cementitious content" to meet strength-development requirements.
- In testing by BASF, the 28-day strength-development for 55% replacement of Portland cement by a natural pozzolanic EMC was 14,000 psi / 96.5 MPa (i.e. > C95). This comprised a "total cementitious content" of 335 kg/m^3 (564 lbs/CY) concrete mix.
EMCs as "low carbon" cements
[edit]Unlike Portland Cement, an EMC's production releases no carbon dioxide whatsoever. This makes EMCs "low carbon cements".[8]
The first cited claims for EMC's CO2-reduction capabilities were made in 1999, when worldwide Portland cement production stood at 1.6 billion tonnes per year.[23][28] From 2011 to 2019, worldwide Portland cement production increased from 3.6 to 4.1 billion tonnes per year.[29][Note 1] Energetically modified cement's potential for contributing to a worldwide reduction of CO2 has been externally recognised since 2002 and has been ongoing.[6][7][9] Recent recognition has included the 2019 Energy Transitions Commission (Lord Adair Turner and Lord Stern) report Mission Possible sectoral focus: cement (2019).[30] Recognition of the "Zero-Carbon" potential was set out by McKinsey & Co in its 2020 report Laying the foundation for zero-carbon cement.[31] In 2023, the contribution offered by EMCs in achieving "low carbon" materials was further acknowledged within the academic domain of mechanochemistry.[17]
Production and field-usage
[edit]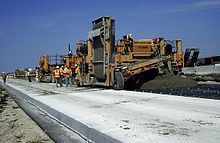
(Interstate Highway), Texas, United States.
No noxious emissions or toxic chemicals during production
[edit]EMC Activation is purely a mechanical process. As such, it does not involve heating or burning or indeed any chemical treatments. This means no fumes at all are produced during an EMC's manufacture.[23]
History
[edit]EMCs have been produced for project usage since 1992 for a wide range of uses.[5] By 2010, the volume of concrete poured containing EMCs was about 4,500,000 cu yd (3,440,496 m3), largely on US DOT projects.[5] To place this into context, that is more than the entire construction of the Hoover Dam, its associated power plants and appurtenant works, where a total of 4,360,000 cu·yds (3,333,459 m3) of concrete was poured—equivalent to a U.S. standard highway from San Francisco to New York City.[32]
Early usage in Sweden
[edit]An early project used a concrete comprising a 50% Portland cement substitution using a silica sand EMC. This was deployed for the construction of a road bridge in Karungi, Sweden, in 1999, with Swedish construction firm Skanska. The Karungi road bridge has withstood Karungi's harsh subarctic climate and divergent annual and diurnal temperature ranges.[23]
Usage in the United States
[edit]In the United States, energetically modified cements have been approved for usage by a number of state transportation agencies, including PennDOT, TxDOT and CalTrans.[25]
In the United States, highway bridges and hundreds of miles of highway paving have been constructed using concretes made from EMC derived from fly ash.[5] These projects include sections of Interstate 10.[5] In these projects, EMC replaced at least 50% of the Portland cement in the concrete poured.[26] This is about 2.5 times more than the typical amount of fly ash in projects where energetic modification is not used.[33] Independent test data showed 28-day strength-development requirements were exceeded in all projects. In 2009, fly ash EMCs were demonstrated to exceed the 'Grade 120 Slag' benchmark per ASTM C989.[26]
Another project was the extension of the passenger terminals at the Port of Houston, Texas, where energetically modified cement's ability to yield concretes that exhibit high resistances to chloride– and sulphate–ion permeability (i.e., increased resistance to seawater) was a factor.[5]
Developments in 2024
[edit]In February 2024 it was jointly announced that a manufacturing plant for EMCs made from volcanic materials will be jointly developed by "EMC Cement" and HES International at the Port of Amsterdam, and further, that the "all-electric zero-emissions plant, of an initial capacity of 1.2 million tonnes, will cut CO2 emissions by 1 million tonnes annually — using less than 10% of the energy of a conventional Portland cement plant".[34]
Properties of concretes and mortars made from EMCs
[edit]
Custom design for end-usage
[edit]The performance of mortars and concretes made from EMCs can be custom-designed. For example, EMC concretes can range from general application (for strength and durability) through to the production of rapid and ultra-rapid hardening high-strength concretes (for example, over 70 MPa / 10,150 psi in 24 hours and over 200 MPa / 29,000 psi in 28 days).[24] This allows energetically modified cements to yield High Performance Concretes.[24]
Durability of EMC concretes and mortars
[edit]Any cementitious material undergoing EMC Activation will likely marshal improved durability—including Portland cement treated with EMC Activation.[24] As regards pozzolanic EMCs, concretes made from pozzolanic EMCs are more durable than concretes made from Portland cement.[36]
Treating Portland cement with EMC activation will yield high-performance concretes (HPCs). These HPCs will be high strength, highly durable, and exhibiting greater strength-development in contrast to HPCs made from untreated Portland cement.[24] Treating Portland cement with the EMC Activation process may increase the strength development by nearly 50% and also significantly improve the durability, as measured according to generally accepted methods.[24][35]
Enhanced resistance to saltwater attack
[edit]Concrete made from ordinary Portland cement without additives has a relatively impaired resistance to saltwater.[35] In contrast, EMCs exhibit high resistances to chloride and sulphate ion attack, together with low alkali-silica reactivities (ASR).[26] For example, durability tests have been performed according to the "Bache method" (see diagram). Samples made of HPC having respective compressive strengths of 180.3 and 128.4 MPa (26,150 and 18,622 psi) after 28 days of curing, were then tested using the Bache method. The samples were made of (a) EMC (comprising Portland cement and silica fume both having undergone EMC Activation) and (b) Portland cement. The resulting mass-loss was plotted in order to determine durability. As a comparison, the test results showed:
- Whereas the reference Portland cement concrete had "total destruction after about 16 Bache method cycles, in line with Bache's own observations for high-strength concrete";[24][35]
- EMC high performance concrete showed a "consistent high-level durability" throughout the entire testing period of 80 Bache cycles, with for example, "practically no scaling of the concrete has been observed".[24]
In other words, treating Portland cement with the EMC Activation process, may increase the strength development by nearly 50% and also significantly improve the durability, as measured according to generally accepted methods.[24]
Low leachability of EMC Concretes
[edit]Leachability tests were performed by LTU in 2001 in Sweden, on behalf of a Swedish power production company, on concrete made from an EMC made from fly ash. These tests confirmed that the cast concrete "showed a low surface specific leachability" with respect to "all environmentally relevant metals."[37][38]
EMCs using Pozzolans such as volcanic materials
[edit]
"self-healing" propensity...
-
PHOTO A [39]
-
PHOTO B
Self-healing properties of pozzolanic EMCs
[edit]Natural pozzolanic reactions can cause mortars and concretes containing these materials to "self-heal".[40][41][42] The EMC Activation process can increase the likelihood of the occurrence of these pozzolanic reactions.[43][44] The same tendency been noted and studied in the various supporting structures of Hagia Sophia built for the Byzantine emperor Justinian (now, Istanbul, Turkey).[45] There, in common with most Roman cements, mortars comprising high amounts of pozzolana were used — in order to give what was thought to be an increased resistance to the stress-effects caused by earthquakes.[46]
EMCs made from pozzolanic materials exhibit "biomimetic" self-healing capabilities that can be photographed as they develop (see picture insert).[39]
EMCs using California pozzolans
[edit]Concretes made by replacing at least 50% of the Portland cement with EMCs have yielded consistent field results in high-volume applications.[26] This is also the case for EMC made from natural pozzolans (e.g., volcanic ash).[47]
Volcanic ash deposits from Southern California were independently tested; at 50% Portland cement replacement, the resulting concretes exceeded the requirements of the relevant US standard.[48] At 28 days, the compressive strength was 4,180 psi / 28.8 MPa (N/mm²). The 56-day strength exceeded the requirements for 4,500 psi (31.1 MPa) concrete, even taking into account the safety margin as recommended by the American Concrete Institute.[49] The concrete made in this way was workable and sufficiently strong, exceeding the 75% standard of pozzolanic activity at both 7 days and 28 days.[48] The surface smoothness of pozzolans in the concrete was also increased.[48]
Effect on pozzolanic reactions
[edit]
EMC Activation is a process that increases a pozzolan's chemical affinity for pozzolanic reactions.[43][44] This leads to faster and greater strength development of the resulting concrete, at higher replacement ratios, than untreated pozzolans.[26][47] These transformed (now highly reactive pozzolans) demonstrate further benefits using known pozzolanic reaction-pathways that typically see as their end-goal a range of hydrated products. An NMR study on EMCs concluded that EMC Activation caused "the formation of thin SiO2 layers around C3S crystals", which in turn, "accelerates the pozzolanic reaction and promotes growing of more extensive nets of the hydrated products".[50]
In simple terms, by using pozzolans in concrete, porous (reactive) Portlandite can be transformed into hard and impermeable (relatively non-reactive) compounds, rather than the porous and soft relatively reactive calcium carbonate produced using ordinary cement.[51] Many of the end products of pozzolanic chemistry exhibit a hardness greater than 7.0 on the Mohs scale."Self healing" capabilities may also contribute to enhanced field-application durabilities where mechanical stresses may be present.
In greater detail, the benefits of pozzolanic concrete, starts with an understanding that in concrete (including concretes with EMCs), Portland cement combines with water to produce a stone-like material through a complex series of chemical reactions, whose mechanisms are still not fully understood. That chemical process, called mineral hydration, forms two cementing compounds in the concrete: calcium silicate hydrate (C-S-H) and calcium hydroxide (Ca(OH)2). This reaction can be noted in three ways, as follows:[52]
- Standard notation:
- Balanced:
- Cement chemist notation (the hyphenation denotes the variable stoichiometry): C3S + H → C-S-H + CH
The underlying hydration reaction forms two products:
- Calcium silicate hydrate (C-S-H), which gives concrete its strength and dimensional stability. The crystal structure of C-S-H in cement paste has not been fully resolved yet and there is still ongoing debate over its nanostructure.[53]
- Calcium hydroxide (Ca(OH)2), which in concrete chemistry is known also as Portlandite. In comparison to calcium silicate hydrate, Portlandite is relatively porous, permeable and soft (2 to 3, on Mohs scale).[54] It is also sectile, with flexible cleavage flakes.[55] Portlandite is soluble in water, to yield an alkaline solution which can compromise a concrete's resistance to acidic attack.[36]
Portlandite makes up about 25% of concrete made with Portland cement without pozzolanic cementitious materials.[51] In this type of concrete, carbon dioxide is slowly absorbed to convert the Portlandite into insoluble calcium carbonate (CaCO3), in a process called carbonatation:[51]
In mineral form, calcium carbonate can exhibit a wide range of hardness depending on how it is formed. At its softest, calcium carbonate can form in concrete as chalk (of hardness 1.0 on Mohs scale). Like Portlandite, calcium carbonate in mineral form can also be porous, permeable and with a poor resistance to acid attack, which causes it to release carbon dioxide.
Pozzolanic concretes, including EMCs, however, continue to consume the soft and porous Portlandite as the hydration process continues, turning it into additional hardened concrete as calcium silicate hydrate (C-S-H) rather than calcium carbonate.[51] This results in a denser, less permeable and more durable concrete.[51] This reaction is an acid-base reaction between Portlandite and silicic acid (H4SiO4) that may be represented as follows:[56]
Further, many pozzolans contain aluminate (Al(OH)4−) that will react with Portlandite and water to form:
- calcium aluminate hydrates, such as calcium aluminium garnet (hydrogrossular: C4AH13 or C3AH6 in cement chemist notation, hardness 7.0 to 7.5 on Mohs scale);[57] or
- in combination with silica, to form strätlingite (Ca2Al2SiO7·8H2O or C2ASH8 in cement chemist notation), which geologically can form as xenoliths in basalt as metamorphosed limestone.[58]
Pozzolanic cement chemistry (along with high-aluminate cement chemistry) is complex and per se is not constrained by the foregoing pathways. For example, strätlingite can be formed in a number of ways, including per the following equation which can add to a concrete's strength:[59][60]
The role of pozzolans in a concrete's chemistry is not fully understood. For example, strätlingite is metastable, which in a high temperature and water-content environment (that can be generated during the early curing stages of concrete) may of itself yield stable calcium aluminium garnet (see first bullet point above).[63] This can be represented per the following equation:
- 3C2AH8 → 2C3AH6 + AH3 + 9H (cement chemist notation) [64]
Per the first bullet point, although the inclusion of calcium aluminium garnet per se is not problematic, if it is instead produced by the foregoing pathway, then micro-cracking and strength-loss can occur in the concrete.[65] However, adding high-reactivity pozzolans into the concrete mix prevents such a conversion reaction.[66] In sum, whereas pozzolans provide a number of chemical pathways to form hardened materials, "high-reactivity" pozzolans such as blast furnace slag (GGBFS) can also stabilise certain pathways. In this context, EMCs made from fly ash have been demonstrated to produce concretes that meet the same characteristics as concretes comprising "120 Slag" (i.e., GGBFS) according to U.S. standard ASTM C989.[26][67]
Portlandite, when exposed to low temperatures, moist conditions and condensation, can react with sulphate ions to cause efflorescence. In contrast, pozzolanic chemistry reduces the amount of Portlandite available, to reduce the proliferation of efflorescence.[68]
EMC Activation
[edit]
EMC Activation's purpose is to cause a fundamental destruction to the crystalline structure of the material processed, to render it amorphous.[43] Although this change increases the processed material's chemical reactivity, no chemical reaction is caused during the EMC Activation process.
At its simplest, mechanochemistry can be stated as "a field studying chemical reactions initiated or accelerated by the direct absorption of mechanical energy."[17] More technically, it can be defined as a branch of chemistry concerned with the "chemical and physico-chemical transformation of substances in all states of aggregation produced by the effect of mechanical energy."[70] IUPAC carries no standard definition of the term mechanochemistry, instead defining a "mechanochemical reaction" as a chemical reaction "induced by the direct absorption of mechanical energy", while noting, "shearing, stretching, and grinding are typical methods for the mechano-chemical generation of reactive sites".[71][72]
More narrowly, "mechanical activation" was a term first defined in 1942 as a process "involving an increase in reaction ability of a substance which remains chemically unchanged."[73] Even more narrowly, EMC Activation is a specialised form of mechanical activation limited to the application of high energy ball milling (HEBM) to cementitious materials. More narrowly than that, EMC Activation uses vibratory milling, and even then, only by using its own grinding media.[43] As stated in a 2023 academic textbook limited to mechanochemistry, EMC Activation has "impressively demonstrated" its effects in causing a change to the reactivity of alternate cement material and the resulting physical characteristics of the concrete cast.[17]
Thermodynamic justification
[edit]More particularly, HEBM can be described as increasing the chemical reactivity of a material by increasing its chemical potential energy. In EMC Activation, transferred mechanical energy is stored in the material as lattice defects caused by destroying the material's crystalline structure. Hence, the process transforms solid substances into thermodynamically and structurally more unstable states, allowing an explanation for that increased reactivity as an increase in Gibbs energy:[74]
- where, for temperature , the terms and are the respective Gibbs values in the processed and unprocessed material.
At its simplest, HEBM causes the destruction of crystalline bonds, to increase a material's reactivity.[75] From the thermodynamic perspective, any subsequent chemical reaction can decrease the excess energy level in the activated-material (i.e. as a reactant) to produce new components comprising both a lower chemical energy and a more stable physical structure. Conversely, to render the pre-processed material into a more reactive physical state, the disordering process during the HEBM process can be justified as being equivalent to a decrystallisation (and hence an entropy increase) that in part yields a volume increase (decrease of bulk density). A reverse process, sometimes called "relaxation", can be almost immediate (10−7 to 10−3 seconds) or take much longer (e.g. 106 seconds).[76] Ultimately, any overall retained thermodynamic effect can be justified on the basis that any such reverse process is incapable of reaching an ideal thermodynamic end-state of its own accord. As a result, in the course of the mechanical activation of minerals, reverse "relaxation" processes cannot completely decrease the Gibbs free energy that has been created. Hence, energy remains in the material, which is stored in the crystal-lattice defects created.[77][78]
Net thermodynamic effect of HEBM
[edit]Overall, HEBM renders a net thermodynamic effect:[79][80][81]
- The structural disordering implies an increase of both entropy and enthalpy and thus stimulates the crystal properties according to the thermodynamic modifications. Only a small fraction (approximately 10%) of the excess enthalpy of the activated product may be accounted-for as surface-area enlargement.
- Instead, the main part of the excess enthalpy and modified properties can mostly be assigned to the development of thermodynamically unstable states in the material's lattice (and not as a reduction of particle size).
- Since the activated system is unstable, the process of activation is reversible—resulting in deactivation, re-crystallization, entropy loss and energy output of system. That reverse ("relaxation") process continues to a thermodynamic equilibrium, but ultimately can never reach an ideal structure (i.e. one free of defects).
- A more complete description of such an "activation" process factors-in enthalpy also, by which according to the Gibbs-Helmholtz equation, the Gibbs free energy between activated and non-activated solid state can be represented:
- where, is the change in enthalpy and the change in entropy.
Resulting crystalline disorder
[edit]Where the crystal disordering is low, is very small (if not negligible). In contrast, in highly deformed and disordered crystals, the values of can have a significant impact on the rendered Gibbs free energy. Leaving aside the heat generated during the process on account of friction etc. occasioned during the activation process, the excess Gibbs free energy retained in the activated material can be justified as being due to two changes, namely an increase in () specific surface area; and () defect structure.[82][81] In successful HEBM processes such as EMC Activation:[83][84]
- as to (), only about 10% of the excess energy of such an activated product may be accounted-for as a change in surface area.
- as to (), almost all the imparted energy is contained in the actual structural defects in the material processed.
An approximation for EMC Activation
[edit]The relatively low value of () as against the high value of () serves to further distinguish HEBM from general grinding or "milling" (where instead the only aim there is to increase the surface area of the materials processed), thereby accounting for an explanation for the change in entropy of the rendered material in the form of elastic energy (stored in lattice defects that can take years to "relax" ) that is the "source of excess Gibbs energy and enthalpy".[82] As for enthalpy , four descriptors can be derived to provide an overview as to the total change during such an activation process:[83][85][86]
- where:
- is a measure of the dislocation density;
- is a measure of new phases (polymorphic transformation);
- is a measure of the formation of amorphous material;
- is a measure of specific surface area.
- where:
Because the majority of the work exacted during the EMC Activation process goes to aspect () above, is trivial. Hence the major functions for the change in enthalpy approximate to:
In EMC Activation, the foregoing terms and are seen as being particularly prominent because of the nature of the changes in the physical structure observed.[43] Hence, the change in enthalpy occasioned during EMC Activation can be approximated to:[85][86]
- i.e,
- where:
- , , and correspond respectively to the molar volume of the material, Burgers vector, shear modulus and dislocation density;[85][86]
- and are respectively the concentration of the amorphous phase and molar amorphisation energy.[85][86]
Low temperature reactivity
[edit]From the above thermodynamic construct, EMC Activation results in a highly amorphous phase that can be justified as a large and also a large increase.[43][85][86] The benefits of the EMC Activation being large in means that an EMC's reactivity is less temperature dependent. In terms of any reaction's thermodynamic impetus, a reactant's overall is not dependent, meaning that a material having undergone HEBM with a corresponding elevation of can react at a lower temperature (as the "activated" reactant is rendered less reliant on the temperature-dependent function for its onward progression). Further, an EMC's reaction can exhibit physical mechanisms at extremely small scales "with the formation of thin SiO2 layers" to aid a reaction's pathway—with the suggestion that EMC Activation increases the ratio of favourable reaction sites.[50] Studies elsewhere have determined that HEBM can significantly lower the temperature required for a subsequent reaction to proceed (up to a three-fold reduction), whereby a major component of the overall reaction-dynamics is initiated at a "nanocrystalline or amorphous phase" to exhibit "unusually low or even negative values of the apparent activation energy" required to cause a chemical reaction to occur.[87]
Overall, EMCs are likely less temperature dependent for a chemical pathway's onward progression (see section above on Pozzolanic reactions), which may explain why EMCs provide self-healing benefits even at low arctic temperatures.[88][89]
Physical justification (amorphisation)
[edit]Large changes in , more particularly in the resultant values of and provide an insight into EMC Activation's efficacy. The amorphisation of crystalline material at high-pressure conditions "is a rather unusual phenomenon" for the simple reason that "most materials actually experience the reverse transformation from amorphous to crystalline at high-pressure conditions".[90] Amorphisation represents a highly distorted "periodicity" of a material's lattice element, comprising a relatively high Gibbs free energy.[77][79] Indeed, amorphisation may be compared to a quasi-molten state.[78][80]
As a possible explanation of why amorphous silica is more reactive than its crystalline version, thermodynamic treatments may give further insight, even if such approaches cannot fully explain the phenomenon. For example, the so-called "glass transition temperature" increases with an increasing cooling rate (i.e., ) that allows energy to be accumulated as if "frozen in".[91] Thus, by substantially increasing that cooling rate, "glasses with thermodynamic properties can be obtained, substantially different from those of the initial metastable undercooled liquid".[92] At very high cooling rates, the enthalpy frozen into the resulting vitrified system can be equal to (or exceed) the enthalpy of melting where the cooling rate is to the order of 106 to 109 K/s and upwards.[93] Hence, assuming that the shock-wave dynamics of the EMC Activation process hold true, such that focal nanoscale temperature fluctuations are extremely transient (as described generally per the next section), means that the cooling rates during the HEBM process are to at least similar orders of magnitude, if not more. Hence:
- (recalling that )
As a result of the rise in , the chemical potential of the system is increased and "frozen in", which gives rise to an increase in any subsequent reactivity. All told, in common with other HEBM processes, EMC Activation causes crystalline destruction because of extremely violent and disruptive factors that are occasioned at the nanoscale of the material being processed.[94] Although over in short duration and highly focal, the processes are repeated at a high frequency: hence those factors are thought to mimic pressures and temperatures found deep inside the Earth to cause the required phase change.[3] For example, Peter Thiessen developed the magma-plasma model that assumes localised temperatures—higher than 103 kelvins—can be generated at the various impact points to induce a momentary excited plasma state in the material, characterized by the ejection of electrons and photons together with the formation of excited fragments (see diagram above).[95] Experimental data gathered from localised crack-generation, itself an important component of EMC Activation, has confirmed temperatures in this region as long ago as 1975.[96]
Vibratory Ball Mills (VBMs)
[edit]For EMC activation, the HEBM method used is a vibratory ball mill (VBM).[43] A VBM uses a vertical eccentric drive-mechanism to vibrate an enclosed chamber up to many hundreds of cycles per minute. The chamber is filled with the material being processed together with specialised objects called grinding media. In their most simple format, such media can be simple balls made from specialised ceramics. In practical terms, EMC Activation deploys a range of grinding media of different sizes, shapes and composites to achieve the required mechanochemical transformation.[5]
It has been suggested that a VBM will grind at 20 to 30 times the rate of a rotary ball mill, reflecting that a VBM's mechanism is especially rapacious.[97]
VBM kinetics
[edit]In simple terms, the compressive force acting between two identical colliding balls in a VBM can be expressed:[98]
- where,
- where, is the mass of both balls, the radius, the absolute velocity of impact and the Young's modulus of the balls' material.[98]
As can be seen, an increase in velocity of impact increases . The size and mass of the grinding media also contribute. 's denominator term incorporates meaning that the nature of the material used for the grinding media is an important factor ( is ultimately squared in , so its negative value is of no consequence). More fundamentally, due to the rapid vibration a high acceleration is imparted to the grinding media, whereupon the continuous, short, sharp impacts on the load result in rapid particle-size reduction.[97] In addition, high pressures and shear stresses facilitate the required phase transition to an amorphous state both at the point of impact and also during the transmission of shock-waves that can yield even greater pressures than the impact itself.[94]
For example, the contact time of a two-ball collision can be as short as 20μs, generating a pressure of 3.3 GPa upwards and with an associated ambient temperature increase of 20 kelvins.[94] Because of the short duration of the impact, the rate of change in momentum is significant—generating a shock wave of duration only 1-100μs but with an associated pressure of 10 GPa upwards and a highly localised and focal temperature (i.e., at the nanoscale) up to several thousands of kelvins.[94] To place this into context, a pressure of 10GPa is equivalent to about 1,000 kilometers of sea water. As a further example, the impact of two identical steel balls of 2.5 cm diameter of velocity 1 m/s will generate a collision energy density of over 109 joules/m2, with alumina balls of the same 2.5 cm diameter and velocity of 1 m/s generating an even greater energy density.[98] The collisions occur in a very short timescale and hence the "rate of energy release over the relatively small contact area can be very high".[98]
See also
[edit]Background science to EMC Activation:
- Contact mechanics – Study of the deformation of solids that touch each other
- Crystallinity – Degree of structural order in a solid
- Crystal structure – Ordered arrangement of atoms, ions, or molecules in a crystalline material
- Hardness – Measure of a material's resistance to localized plastic deformation
- Lattice constant – Physical dimensions of unit cells in a crystal
- Material mechanics – Behavior of solid objects subject to stresses and strains
- Materials science – Research of materials
- Microstructure – Very small scale structure of material
- Peter Adolf Thiessen – German physical chemist (1899–1990)
- Surface engineering – Altering the properties of solid surfaces
- Surface metrology – Measurement of small-scale features on surfaces
- Tribology – Science and engineering of interacting surfaces in relative motion
Academic:
- Luleå University of Technology – University in Sweden
Notes
[edit]- ^ Two aspects: (I) 2011 Global Portland cement production was approximately 3.6 billion tonnes per United States Geological Survey (USGS) (2013) data, and is binding as a reasonably accurate assimilation, rather than an estimate per se. Note also, that by the same report, for 2012 it was estimated that Global Portland cement production would increase to 3.7 billion tonnes (a 100 million tonne increase, year-on-year), when in fact the actual figure for 2012 was 3.8 billion tonnes. (II) 2011 Estimate of Global total CO2 production: 33.376 billion tonnes (without international transport). Source: E.U. European Commission, Joint Research Centre (JRC)/PBL Netherlands Environmental Assessment Agency. Emission Database for Global Atmospheric Research (EDGAR), release version 4.2. The 2009–2011 trends were estimated for energy-related sectors based on fossil fuel consumption for 2009–2011 from the BP Review of World Energy 2011 (BP, 2012), for cement production based on preliminary data from USGS (2012), except for China for which use was made of National Bureau of Statistics of China (NBS) (2009, 2010, 2011).
[As of May 2013. See, EDGAR, external link section]. - ^ The "Bache method" for testing concrete durability simulates daily temperature variations in brine. Test 1 or Test 2 may be used, or performed sequentially over 48hrs. The method induces saturation by 7.5% salt water (i.e., higher concentration than sea waters), followed by freezing or heating in a 24-hour cycle to simulate high diurnal temperature ranges. The chosen cycle is repeated ad nausem to determine the mass-loss. Hence, the Bache method is generally accepted as one of the most severe testing procedures for concrete as an analogue for durability.
- ^ The large photo depicts a concrete test-beam made from an EMC undergoing RILEM 3-point bending at Luleå University of Technology in Sweden (Feb., 2013). This treatment induces cracks to test for "self-healing" propensities. Concrete (total cmt: 350 kg/m³) containing 40% Portland cement and 60% EMC made from fly ash was used. PHOTO A: Cracks of average width 150-200 μm were induced after circa 3-weeks' water-curing. PHOTO B: Without any intervention, the high volume pozzolan concrete exhibited the gradual filling-in of the cracks with newly-synthesized CSH gel (a product of the ongoing pozzolanic reaction). These were completely filled-in after ~4.5 months. During the observation period, continuous strength-development was also recorded by virtue of the ongoing pozzolanic reaction. This, together with the observed "self healing" properties, have a positive impact on concrete durability. All photos Dr. V. Ronin and The Nordic Concrete Federation.
- ^ Further notes on pozzolanic chemistry: (A) The ratio Ca/Si (or C/S) and the number of water molecules can vary, to vary C-S-H stoichiometry. (B) Often, crystalline hydrates are formed for example when tricalcium aluminiate reacts with dissolved calcium sulphate to form crystalline hydrates (3CaO·(Al,Fe)2O3·CaSO4·nH2O, general simplified formula). This is called an AFm ("alumina, ferric oxide, monosulphate") phase. (C) The AFm phase per se is not exclusive. On the one hand while sulphates, together with other anions such as carbonates or chlorides can add to the AFm phase, they can also cause an AFt phase where ettringite is formed (6CaO·Al2O3·3SO3·32H2O or C6S3H32). (D) Generally, the AFm phase is important in the further hydration process, whereas the AFt phase can be the cause of concrete failure known as DEF. DEF can be a particular problem in non-pozzolanic concretes (see, for ex., Folliard, K., et al., Preventing ASR/DEF in New Concrete: Final Report, TXDOT & U.S. FHWA:Doc. FHWA/TX-06/0-4085-5, Rev. 06/2006). (E) It is thought that pozzolanic chemical pathways utilising Ca2+ ions cause the AFt route to be relatively suppressed.
References
[edit]- ^ Mark Anthony Benvenuto (24 February 2015). Industrial Chemistry: For Advanced Students. De Gruyter. pp. 134–. ISBN 978-3-11-035170-5.
- ^ Pentimalli, M; Bellusci, M; Padella, F (2015). Aliofkhazraei, M (ed.). "High-Energy Ball Milling as a General Tool for Nanomaterials Synthesis and Processing". Handbook of Mechanical Nanostructuring: 663–679. doi:10.1002/9783527674947.ch28. ISBN 978-3-527-33506-0.
- ^ a b c Tole, Ilda; Habermehl-Cwirzen, Karin; Cwirzen, Andrzej (1 August 2019). "Mechanochemical activation of natural clay minerals: an alternative to produce sustainable cementitious binders – review". Mineralogy and Petrology. 113 (4). Springer: 449–462. Bibcode:2019MinPe.113..449T. doi:10.1007/s00710-019-00666-y.
- ^ a b Jean-Pierre Bournazel; Yves Malier (1998). PRO 4: International RILEM Conference on Concrete: From Material to Structure. RILEM Publications. pp. 101–. ISBN 978-2-912143-04-4.
- ^ a b c d e f g h Ronin, V (2010). An Industrially Proven Solution for Sustainable Pavements of High-Volume Pozzolan Concrete – Using Energetically Modified Cement, EMC (PDF). Washington DC, United States: Transportation Research Board of the National Academies.
- ^ a b Humpreys, K.; Mahasenan, M. (2002). Toward a Sustainable Cement Industry Substudy 8: Climate Change. Geneva, Switzerland: World Business Council for Sustainable Development (WBCSD).
- ^ a b c Harvey, D (2013). Energy and the New Reality 1 – Energy Efficiency and the Demand for Energy Services. Taylor & Francis. ISBN 9781136542718.
- ^ a b Kumar, Rakesh; Kumar, Sanjay; Mehrotra, S.P. (December 2007). "Towards sustainable solutions for fly ash through mechanical activation". Resources, Conservation and Recycling. 52 (2): 157–179. Bibcode:2007RCR....52..157K. doi:10.1016/j.resconrec.2007.06.007.
- ^ a b Hasanbeigi, Ali; Price, Lynn; Lin, Elina (October 2012). "Emerging energy-efficiency and CO2 emission-reduction technologies for cement and concrete production: A technical review". Renewable and Sustainable Energy Reviews. 16 (8): 6220–6238. doi:10.1016/j.rser.2012.07.019. S2CID 108511643.
- ^ Hickenboth, Charles R.; Moore, Jeffrey S.; White, Scott R.; Sottos, Nancy R.; Baudry1, Jerome; Wilson, Scott R. (2007). "Biasing Reaction Pathways with Mechanical Force". Nature. 446 (7134): 423–427. Bibcode:2007Natur.446..423H. doi:10.1038/nature05681. PMID 17377579. S2CID 4427747.
{{cite journal}}
: CS1 maint: numeric names: authors list (link)(subscription required) - ^ Carlier, Leslie; Baron, Michel; Chamayou, Alain; Couarraze, Guy (May 2013). "Greener pharmacy using solvent-free synthesis: Investigation of the mechanism in the case of dibenzophenazine" (PDF). Powder Technology. 240: 41–47. doi:10.1016/j.powtec.2012.07.009. S2CID 97605147.
- ^ Banerjee, S; Mukhopadhyay, P (2007). "Solidification, Vitrification, Crystallization and Formation of Quasicrystalline and Nanocrystalline Structures". Pergamon Materials Series. 12: 122–255. doi:10.1016/S1470-1804(07)80056-3. ISBN 978-0-08-042145-2. ISSN 1470-1804.
- ^ Živanović, Deana; Andrić, Ljubiša; Sekulić, Živko; Milošević, Siniša (1999). "Mechanical Activation of Mica". Advanced Science and Technology of Sintering. pp. 211–217. doi:10.1007/978-1-4419-8666-5_29. ISBN 978-1-4613-4661-6.
- ^ Danny Harvey (12 August 2010). Energy and the New Reality 1:Energy Efficiency and the Demand for Energy Services. Routledge. pp. 385–. ISBN 978-1-136-54272-5.
- ^ Eflgren, Lennart (27 March 2013). "Future Infrastructure Forum: Scandinavian Points of View" (PDF). Future Infrastructure Forum, Cambridge University.
- ^ "Stipendieutdelning" (in Swedish). Luleå tekniska universitet. 30 August 2006. Retrieved 24 March 2014.
- ^ a b c d Colacino, Evelina; Garcia, Felipe (2023). Mechanochemistry and Emerging Technologies for Sustainable Chemical Manufacturing (1st ed.). Routledge. p. 162. ISBN 9780367775018.
- ^ Ronin, V.; Jonasson, J.E. "New concrete technology with the use of energetically modified cement (EMC)". Proceedings: Nordic Concrete Research Meeting: Göteborg 1993. Oslo, Norway: Norsk Betongforening (Nordic concrete research): 53–55. ISSN 0800-6377.
- ^ LTU website (27 January 2024). "Professor Lennart Elfgren". ltu.se.
- ^ LTU website. "The Vice-Chancellor's Medal for Merit awarded to Lennart Elfgren". ltu.se.
- ^ EUREKA. "EUREKA Gold Award for EMC Cement" (PDF).
- ^ Krishnaraj, L; Reddy, YBS; Madhusudhan, N; Ravichandran, PT (2017). "Effect of energetically modified Fly Ash on the durability properties of cement mortar". Rasayan Journal of Chemistry. 10 (2): 423–428. doi:10.7324/RJC.2017.1021682.
- ^ a b c d Hedlund, H; Ronin, V; Jonasson, J.E.; Elfgren, L (1999). "Grönare Betong" [Green Cement]. Bygg & Teknik (in Swedish). 91 (7). Stockholm, Sweden: Förlags AB Bygg & teknik: 12–13. ISSN 2002-8350.
- ^ a b c d e f g h i Elfgren, L; Justnes, H; Ronin, V (2004). High Performance Concretes With Energetically Modified Cement (EMC) (PDF). Kassel, Germany: Kassel University Press GmbH. pp. 93–102.
- ^ a b United States Federal Highway Administration (FHWA). "EMC Cement Presentation January 18, 2011". Washington, DC.
- ^ a b c d e f g EMC Cement BV. Summary of CemPozz® (Fly Ash) Performance in Concrete (PDF). EMC Cement BV, 2012.
- ^ "Low Carbon Cement Homepage". An official website of EMC Cement. EMC Cement. Retrieved 9 June 2024.
- ^ "Cement Data Sheet" (PDF). U.S. Geological Survey. USGS. 2001. Retrieved 14 August 2020.
- ^ "Cement Data Sheet" (PDF). U.S. Geological Survey. USGS. 2020. Retrieved 10 August 2020.
- ^ Energy Transitions Commission; Stern, Nicholas; Turner, Adair (2019). "Mission Possible sectoral focus: cement". Energy Transitions Commission. p. 15. Retrieved 1 January 2022.
- ^ Czigler, T; Reiter, S; Somers, K (May 2020). "Laying the foundation for zero-carbon cement". McKinsey & Co. Archived from the original on 16 September 2020. Retrieved 24 August 2020.
{{cite web}}
: CS1 maint: bot: original URL status unknown (link) - ^ USBR. "Hoover Dam Frequently Asked Questions and Answers". U.S. Bureau of Reclamation. Retrieved 10 August 2020.
- ^ Schneider, M.; Romer M., Tschudin M. Bolio C.; Tschudin, M.; Bolio, H. (2011). "Sustainable cement production – present and future". Cement and Concrete Research. 41 (7): 642–650. doi:10.1016/j.cemconres.2011.03.019.
- ^ EMC Cement (26 February 2024). "BREAKTHROUGH ZERO-CARBON CEMENT PLANT TO BE BUILT AT THE PORT OF AMSTERDAM". Low Carbon Cement. Retrieved 29 February 2024.
- ^ a b c d Bache, M (1983). "Densified cement/ultra fine particle-based materials". Proceedings of the Second International Conference on Superplasticizers in Concrete.
- ^ a b Chappex, T.; Scrivener K. (2012). "Alkali fixation of C-S-H in blended cement pastes and its relation to alkali silica reaction". Cement and Concrete Research. 42 (8): 1049–1054. doi:10.1016/j.cemconres.2012.03.010.
- ^ Private study, Luleå University of Technology (2001) "Diffusionstest för cementstabiliserad flygaska", LTU Rapport AT0134:01, 2001-09-03
- ^ Ronin, V; Jonasson, J-E; Hedlund, H (1999). "Ecologically effective performance Portland cement-based binders", proceedings in Sandefjord, Norway 20–24 June 1999. Norway: Norsk Betongforening. pp. 1144–1153.
- ^ a b Ronin, Vladimir; Emborg, Mats; Elfgren, Lennart (2014). "Self-Healing Performance and Microstructure Aspects of Concrete Using Energetically Modified Cement with a High Volume of Pozzolans". Nordic Concrete Research. 51: 131–144 https://www.diva-portal.org/smash/record.jsf?dswid=-9911&pid=diva2%3A987595.
- ^ Yang, Yingzi; Lepech, Michael D.; Yang, En-Hua; Li, Victor C. (May 2009). "Autogenous healing of engineered cementitious composites under wet–dry cycles". Cement and Concrete Research. 39 (5): 382–390. doi:10.1016/j.cemconres.2009.01.013.
- ^ Li, Victor C.; Herbert, Emily (28 June 2012). "Robust Self-Healing Concrete for Sustainable Infrastructure". Journal of Advanced Concrete Technology. 10 (6): 207–218. doi:10.3151/jact.10.207. hdl:2027.42/94191.
- ^ Van Tittelboom, Kim; De Belie, Nele (27 May 2013). "Self-Healing in Cementitious Materials—A Review". Materials. 6 (6): 2182–2217. Bibcode:2013Mate....6.2182V. doi:10.3390/ma6062182. PMC 5458958. PMID 28809268.
- ^ a b c d e f g Justnes, Harald; Elfgren, Lennart; Ronin, Vladimir (February 2005). "Mechanism for performance of energetically modified cement versus corresponding blended cement". Cement and Concrete Research. 35 (2): 315–323. doi:10.1016/j.cemconres.2004.05.022.
- ^ a b Patent abstract for granted patent "Process for Producing Blended Cements with Reduced Carbon Dioxide Emissions" (Pub. No.:WO/2004/041746; International Application No.: PCT/SE2003001009; Pub. Date: 21.05.2004; International Filing Date: 16.06.2003)
- ^ Moropoulou, A.; Cakmak, A.; Labropoulos, K.C.; Van Grieken, R.; Torfs, K. (January 2004). "Accelerated microstructural evolution of a calcium-silicate-hydrate (C-S-H) phase in pozzolanic pastes using fine siliceous sources: Comparison with historic pozzolanic mortars". Cement and Concrete Research. 34 (1): 1–6. doi:10.1016/S0008-8846(03)00187-X.
- ^ Moropoulou, A.; Cakmak, A.S.; Biscontin, G.; Bakolas, A.; Zendri, E. (December 2002). "Advanced Byzantine cement based composites resisting earthquake stresses: the crushed brick/lime mortars of Justinian's Hagia Sophia". Construction and Building Materials. 16 (8): 543–552. doi:10.1016/S0950-0618(02)00005-3.
- ^ a b EMC Cement BV. Summary of CemPozz® (Natural Pozzolan) Performance in Concrete (PDF). EMC Cement BV, 2012.
- ^ a b c Stein, B (2012). A Summary of Technical Evaluations & Analytical Studies of Cempozz® Derived from Californian Natural Pozzolans (PDF). San Francisco, United States: Construction Materials Technology Research Associates, LLC.
- ^ ACI 318 "Building Code Requirements for Structural Concrete and Commentary"
- ^ a b Johansson, K; Larrson, C; Antzutkin, O; Forsling, W; Rao, KH; Ronin, V (1999). "Kinetics of the hydration reactions in the cement paste with mechanochemically modified cement 29Si magic-angle-spinning NMR study". Cement and Concrete Research. 29 (10). Pergamon: 1575–81. doi:10.1016/S0008-8846(99)00135-0. Retrieved 14 August 2020.
- ^ a b c d e Baroghel Bouny, V (1996). Bournazel, J. P.; Malier, Y. (eds.). Texture and Moisture Properties of Ordinary and High Performance Cementitious Materials (in PRO 4: Concrete: From Material to Structure). 144 at 156: RILEM. p. 360. ISBN 2-912143-04-7.
{{cite book}}
: CS1 maint: location (link) - ^ "Cement hydration". Understanding Cement.
- ^ Thomas, Jeffrey J.; Jennings, Hamlin M. (January 2006). "A colloidal interpretation of chemical aging of the C-S-H gel and its effects on the properties of cement paste". Cement and Concrete Research. 36 (1): 30–38. doi:10.1016/j.cemconres.2004.10.022.
- ^ "Portlandite Mineral Data". www.webmineral.com.
- ^ "Handbook of Mineralogy" (PDF). rruff.geo.arizona.edu.
- ^ Mertens, G.; Snellings, R.; Van Balen, K.; Bicer-Simsir, B.; Verlooy, P.; Elsen, J. (March 2009). "Pozzolanic reactions of common natural zeolites with lime and parameters affecting their reactivity". Cement and Concrete Research. 39 (3): 233–240. doi:10.1016/j.cemconres.2008.11.008.
{{cite journal}}
: CS1 maint: multiple names: authors list (link) - ^ Ca3Al2(SiO4)3−x(OH)4x, with hydroxide (OH) partially replacing silica (SiO4)
- ^ Webmineral.com. "Stratlingite Mineral Data". Retrieved 6 December 2013.. See, also, Ding, Jian; Fu, Yan; Beaudoin, J.J. (August 1995). "Strätlingite formation in high alumina cement – silica fume systems: Significance of sodium ions". Cement and Concrete Research. 25 (6): 1311–1319. doi:10.1016/0008-8846(95)00124-U.
- ^ Midgley, H.G.; Bhaskara Rao, P. (March 1978). "Formation of stratlingite, 2CaO.SiO2.Al2O3.8H2O, in relation to the hydration of high alumina cement". Cement and Concrete Research. 8 (2): 169–172. doi:10.1016/0008-8846(78)90005-4.
- ^ Midgley, H.G. (March 1976). "Quantitative determination of phases in high alumina cement clinkers by X-ray diffraction". Cement and Concrete Research. 6 (2): 217–223. doi:10.1016/0008-8846(76)90119-8.
- ^ Heikal, M.; Radwan, M M; Morsy, M S (2004). "Influence of curing temperature on the Physico-mechanical, Characteristics of Calcium Aluminate Cement with air cooled Slag or water cooled Slag" (PDF). Ceramics-Silikáty. 48 (4): 185–196.
- ^ Abd-El.Aziz, M.A.; Abd.El.Aleem, S.; Heikal, Mohamed (January 2012). "Physico-chemical and mechanical characteristics of pozzolanic cement pastes and mortars hydrated at different curing temperatures". Construction and Building Materials. 26 (1): 310–316. doi:10.1016/j.conbuildmat.2011.06.026.
- ^ Mostafa, Nasser Y.; Zaki, Z.I.; Abd Elkader, Omar H. (November 2012). "Chemical activation of calcium aluminate cement composites cured at elevated temperature". Cement and Concrete Composites. 34 (10): 1187–1193. doi:10.1016/j.cemconcomp.2012.08.002.
- ^ Taylor, HFW, (1990) Cement chemistry, London: Academic Press, pp.319–23.
- ^ Matusinović, T; Šipušić, J; Vrbos, N (November 2003). "Porosity–strength relation in calcium aluminate cement pastes". Cement and Concrete Research. 33 (11): 1801–1806. doi:10.1016/S0008-8846(03)00201-1.
- ^ Majumdar, A.J.; Singh, B. (November 1992). "Properties of some blended high-alumina cements". Cement and Concrete Research. 22 (6): 1101–1114. doi:10.1016/0008-8846(92)90040-3.
- ^ ASTM International (2010). "ASTM C989: Standard Specification for Slag Cement for Use in Concrete and Mortars". Book of Standards Volume. 4 (2). doi:10.1520/c0989-10.
- ^ Nhar, H.; Watanabe, T.; Hashimoto, C. & Nagao, S. (2007). Efflorescence of Concrete Products for Interlocking Block Pavements (Ninth CANMET/ACI International Conference on Recent Advances in Concrete Technology: Editor, Malhotra, V., M., 1st ed.). Farmington Hills, Mich.: American Concrete Institute. pp. 19–34. ISBN 9780870312359.
- ^ Boldyrev, V.V.; Pavlov, S.V.; Goldberg, E.L. (March 1996). "Interrelation between fine grinding and mechanical activation". International Journal of Mineral Processing. 44–45: 181–185. Bibcode:1996IJMP...44..181B. doi:10.1016/0301-7516(95)00028-3.
- ^ Heinicke, G.; Hennig, H.-P.; Linke, E.; Steinike, U.; Thiessen, K.-P.; Meyer, K. (1984). "Tribochemistry: In Co-Operation with H.P. Hennig, et al" [and with a preface by Peter-Adolf Thiessen]. Acta Polymerica. 36 (7). Berlin: Akademie-Verlag: 400–401. doi:10.1002/actp.1985.010360721.
- ^ IUPAC, Compendium of Chemical Terminology, 2nd ed. (the "Gold Book") (1997). Online corrected version: (2006–) "Mechano-chemical reaction". doi:10.1351/goldbook.MT07141
- ^ Baláž, Peter; Achimovičová, Marcela; Baláž, Matej; Billik, Peter; Cherkezova-Zheleva, Zara; Criado, José Manuel; Delogu, Francesco; Dutková, Erika; Gaffet, Eric; Gotor, Francisco José; Kumar, Rakesh; Mitov, Ivan; Rojac, Tadej; Senna, Mamoru; Streletskii, Andrey; Wieczorek-Ciurowa, Krystyna (2013). "Hallmarks of mechanochemistry: from nanoparticles to technology". Chemical Society Reviews. 42 (18): 7571–8137. doi:10.1039/c3cs35468g. hdl:10261/96958. PMID 23558752. S2CID 205853500.
- ^ Smekal, A. (April 1942). "Ritzvorgang und molekulare Festigkeit". Die Naturwissenschaften. 30 (14–15): 224–225. Bibcode:1942NW.....30..224S. doi:10.1007/BF01481226. S2CID 1036109.
- ^ Hüttig, Gustav F. (1943). "Zwischenzustände bei Reaktionen im festen Zustand und ihre Bedeutung für die Katalyse". Heterogene Katalyse III. pp. 318–577. doi:10.1007/978-3-642-52046-4_9. ISBN 978-3-642-52028-0.
- ^ Zelikman, AN; Voldman, GM; Beljajevskaja, LV (1975). Teorija Gidrometalurgiceskich Processov [Theory of hydrometallurgical processes] (in Russian). Metallurgija.
- ^ Meyer, K (1968). Physikalisch-chemische Kristallographie. VEB Deutscher Verlag für Grundstoffindustrie. p. 337. ASIN B0000BSNEK.
- ^ a b Pourghahramani, P; Forssberg, E (March 2007). "Effects of mechanical activation on the reduction behavior of hematite concentrate". International Journal of Mineral Processing. 82 (2): 96–105. Bibcode:2007IJMP...82...96P. doi:10.1016/J.MINPRO.2006.11.003.
- ^ a b Pourghahramani, P; Forssberg, E (March 2007). "Reduction kinetics of mechanically activated hematite concentrate with hydrogen gas using nonisothermal methods". Thermochimica Acta. 454 (2): 69–77. Bibcode:2007TcAc..454...69P. doi:10.1016/j.tca.2006.12.023.
- ^ a b Pourghahramani, P; Forssberg, E (May 2006). "Comparative study of microstructural characteristics and stored energy of mechanically activated hematite in different grinding environments". International Journal of Mineral Processing. 79 (2): 120–139. Bibcode:2006IJMP...79..120P. doi:10.1016/j.minpro.2006.01.010.
- ^ a b Pourghahramani, P; Forssberg, E (May 2006). "Microstructure characterization of mechanically activated hematite using XRD line broadening". International Journal of Mineral Processing. 79 (2): 106–119. Bibcode:2006IJMP...79..106P. doi:10.1016/j.minpro.2006.02.001.
- ^ a b Pourghahramani, P; Forssberg, E (September 2007). "Changes in the structure of hematite by extended dry grinding in relation to imposed stress energy". Powder Technology. 178 (1): 30–39. doi:10.1016/j.powtec.2007.04.003.
- ^ a b Pourghahramani, P (2007). Mechanical Activation of Hematite Using Different Grinding Methods with Special Focus on Structural Changes and Reactivity (PDF) (Thesis). p. 242.
- ^ a b Tkáčová, K.; Baláž, P.; Mišura, B.; Vigdergauz, V.E.; Chanturiya, V.A. (July 1993). "Selective leaching of zinc from mechanically activated complex Cu-Pb-Zn concentrate". Hydrometallurgy. 33 (3): 291–300. doi:10.1016/0304-386X(93)90068-O.
- ^ Baláž, P (2000). Extractive metallurgy of activated minerals. Amsterdam: Elsevier Science B.V. p. 292. ISBN 9780080531533. Retrieved 21 August 2020.
- ^ a b c d e Tkáčová, K. (1989). Mechanical activation of minerals. Amsterdam: Elsevier. p. 170. ISBN 978-0444988287.
- ^ a b c d e Tromans, D.; Meech, J.A. (November 2001). "Enhanced dissolution of minerals: stored energy, amorphism and mechanical activation". Minerals Engineering. 14 (11): 1359–1377. Bibcode:2001MiEng..14.1359T. doi:10.1016/S0892-6875(01)00151-0.
- ^ Nepapushev, A. A.; Kirakosyan, K. G.; Moskovskikh, D. O.; Kharatyan, S. L.; Rogachev, A. S.; Mukasyan, A. S. (2015). "Influence of high-energy ball milling on reaction kinetics in the Ni-Al system: An electrothermorgaphic study". International Journal of Self-Propagating High-Temperature Synthesis. 24 (1): 21–28. doi:10.3103/S1061386215010082. S2CID 136668210.
- ^ Ronin, V; Jonasson, JE (1994). Investigation of the effective winter concreting with the usage of energetically modified cement (EMC) - material science aspects, Report 1994:03, 24 pp (in Swedish). Luleå University (LTU), Div. of Struct Eng.
- ^ Ronin, V; Jonasson, JE (1995). High strength and high performance concrete with use of EMC hardening at cold climate conditions. Proceedings of International Conference on Concrete under Severe Conditions, Sapporo, Japan, Luleå University (LTU), Div. of Struct Eng.
- ^ Handle, Philip H.; Loerting, Thomas (2015). "Temperature-induced amorphisation of hexagonal ice". Physical Chemistry Chemical Physics. 17 (7): 5403–5412. Bibcode:2015PCCP...17.5403H. doi:10.1039/C4CP05587J. PMID 25613472. Retrieved 21 August 2020.
- ^ Shi, Frank G. (1994-07-01). "Glass transition: A unified treatment". Journal of Materials Research. 9 (7): 1908–1916. Bibcode:1994JMatR...9.1908S. doi:10.1557/JMR.1994.1908. ISSN 2044-5326. S2CID 137825323.
- ^ Gutzow, Ivan; Todorova, Snejana (2010-04-20). "Glasses as systems with increased solubility, high chemical reactivity and as sources of accumulated energy". Physics and Chemistry of Glasses - European Journal of Glass Science AndTechnology Part B. 51 (2): 83–99.
- ^ Gutzow, Iwan; Schmelzer, J. Jürn; Gutzow, Ivan S. (2013). The vitreous state: thermodynamics, structure, rheology, and crystallization (2nd ed.). Berlin New York: Springer. ISBN 978-3-642-34633-0.
- ^ a b c d Sobolev, Konstantin (August 2005). "Mechano-chemical modification of cement with high volumes of blast furnace slag". Cement and Concrete Composites. 27 (7–8): 848–853. doi:10.1016/j.cemconcomp.2005.03.010.
- ^ Weichert, R.; Schönert, K. (1974). "On the temperature rise at the tip of a fast running crack†". Journal of the Mechanics and Physics of Solids. 22 (2): 127–133. Bibcode:1974JMPSo..22..127W. doi:10.1016/0022-5096(74)90018-0.
- ^ Fuller, K. N. G.; Fox, P. G.; Field, J. E. (1975). "The Temperature Rise at the Tip of Fast-Moving Cracks in Glassy Polymers". Proceedings of the Royal Society of London. Series A, Mathematical and Physical Sciences. 341 (1627): 537–557. Bibcode:1975RSPSA.341..537F. doi:10.1098/rspa.1975.0007. JSTOR 78609. S2CID 137104796.
- ^ a b Krycer, Ian; Hersey, John A. (November 1980). "A comparative study of comminution in rotary and vibratory ball mills". Powder Technology. 27 (2): 137–141. doi:10.1016/0032-5910(80)85015-7.
- ^ a b c d Venkataraman, K.S.; Narayanan, K.S. (May 1998). "Energetics of collision between grinding media in ball mills and mechanochemical effects". Powder Technology. 96 (3): 190–201. doi:10.1016/S0032-5910(97)03368-8.
External links
[edit]- Official website for EMC Cement, Sweden – at lowcarboncement.com
- Luleå University of Technology, Sweden – at LTU.se
- Future Infrastructure Forum, University of Cambridge, United Kingdom – at Fif.construction.cam.ac.uk
- U.S. Geological Survey (USGS) Cement Statistics and Information – at Minerals.usgs.gov
- U.S. Environmental Protection Agency (EPA), Rule Information for Portland Cement Industry – at EPA.gov
- American Concrete Institute – at Concrete.org
- EDGAR – Emission Database for Global Atmospheric Research – at Edgar.jrc.ec.europa.eu
- Vitruvious: The Ten Books on Architecture online: cross-linked Latin text and English translation
- WBCSD Cement Sustainability Initiative Archived 2011-12-18 at the Wayback Machine – at Wbcsdcement.org